By Leah Ellis, PhD and Dr. Yet-Ming Chiang
Can you make zero-carbon cement?
That is the question that launched Sublime’s journey in 2018. We were, and basically still are, battery scientists. If you work on batteries, you de factowork on climate. It was plain to us, and anyone else working on climate solutions, that recent innovations and cost breakthroughs in solar panels, wind turbines, and lithium-ion batteries created a clear path for decarbonization of the energy sector (currently more than 30% of global CO₂). Now the question was how to decarbonize the other 70%.
That’s how two battery scientists started working on cement, which by itself is responsible for about 8% of global CO₂ emissions. It’s one of the single biggest prizes in the trophy room of climate solutions. Cement is the key ingredient in concrete, an essential building material that is literally the foundation of our built environment and the most prolific man-made material in the world. We have relied on cement and concrete for thousands of years. Witness your favorite Roman tourist attractions. Today we make more of it than ever, and we’re just getting started. It is estimated that 75% of the infrastructure that will be in place by 2050 has yet to be built.
How can we reconcile the growth in CO₂-intensive cement production with the global imperative to zero out emissions by 2050? Cement is made in massive ovens that burn at over 1400 °C, temperatures you can only achieve by burning things like coal and gas. When we started, our hunch was that we could replace the big ovens and fossil fuels with electricity and new chemistry to make cement that was at least as strong and no more expensive than the stuff flowing out of kilns today. If we were right, we could ride the massive waves of cheap and abundant renewable electricity and accelerate progress in electrochemistry to meet the world’s rising needs. The two of us and our expanding team of chemists, engineers, and materials scientists have been working to prove that hunch for the past few years. Today, we’re running a system that churns out a ton per month of strong, fast-setting, cost-competitive, clean cement from our lab in Somerville, MA. To help us unlock the next scale-up, we raised a $40m Series A round led by Lowercarbon Capital, Energy Impact Partners, The Engine, Prime Coalition, and cement industry partner Siam Cement Group. (If this is a mission you can get behind, we’re hiring.)
The key to making strong, durable, fast-setting cement is chemistry.
Cement-making technology has changed throughout the past millennia, but the essential formula of hardened cement has remained the same: calcium silicate hydrate, or C-S-H in cement chemists’ shorthand — a material that contains lime (CaO), silica (SiO₂), and water (H₂O). Cement chemistry is complicated (and fascinating!), but ultimately, C-S-H is the most important phase that has given hardened cement its superior strength and durability from ancient Rome through today.
The method of making C-S-H has changed over the years to meet our evolving needs.
Until 200 years ago, cement was made by burning limestone to make lime (1) and mixing that lime with water and amorphous silicates, such as volcanic ash and burnt clays (2):
(1) CaCO₃ + heat (900°C) → CaO + CO₂
(2) CaO + x SiO₂ + y H₂O → CaO (SiO₂)ₓ (H₂O)ᵧ (C-S-H)
Ancient cement was strong and durable, but its wet properties varied with the type of amorphous silicates used, and it didn’t gain strength in the first 3–7 days as quickly as ordinary portland cement (OPC). Invented in the 1800s, OPC soon became the building material of choice during the industrial revolution.
The invention of portland cement was enabled by kilns and fuels that could achieve 1400 °C and the fortuitous discovery of OPC’s chemistry. Portland cement is made by heating a specific ratio of limestone, silica, alumina, and iron. First, it is heated to 900°C to make lime (1) and then to 1400 °C, the temperature at which lime and silica react within a metal oxide flux to form the characteristic phase within portland cement — a tricalcium silicate called alite. To preserve alite — which is thermodynamically stable only above 1250°C — the semi-molten mix is quenched with cool air and ground with gypsum to form a fine cement powder, the form in which it is stored.
When OPC is ready to use, water is added to the cement powder to initiate cement hydration reactions. Alite reacts with water quickly to form C-S-H. To recap: OPC is made by heating limestone and other minerals to above 1400 °C using fossil fuels. The OPC powder is mixed with water, sand, and gravel to make concrete for building applications.
OPC is not an energy-efficient pathway to C-S-H.
The reaction of alite with water is very fast, which gives OPC its fast-setting characteristics and high early strength, but it is also highly exothermic. This is inefficient because the heat of hydration given off is low-grade heat — which is not useful — whereas a large amount of high-grade heat is consumed to make the OPC, a fully dehydrated material, in the first place. Furthermore, OPC hydration creates not just C-S-H but also calcium hydroxide or hydrated lime:
(3) 3CaO SiO₂ + (3+x–y)H₂O → yCaO SiO₂ xH₂O (C-S-H) + (3-y)Ca(OH)₂
This extra hydrated lime does not contribute to hardened cement’s strength. Pure OPC is not optimized for ultimate strength or durability because it does not maximize C-S-H production.
In recent decades, the practice of supplementing OPC with amorphous silicates, also known as supplementary cementitious materials (SCMs), has gained popularity. These added silicates can react with the extra hydrated lime to create more C-S-H through reaction (4), which is very similar to the one that occurred in ancient cement, as described above (2):
(4) Ca(OH)₂ + x SiO₂ + y H₂O → CaO (SiO₂)ₓ (H₂O)(y + 1) (C-S-H)
SCM use in concrete was first adopted to cut costs. Amorphous silicates, particularly those that are common industrial wastes such as coal fly ash, are less expensive than highly processed OPC. They also improve cement’s density and durability because they consume free lime and maximize C-S-H formation.
Eventually, it became clear that “diluting” OPC with SCMs was a low-hanging fruit decarbonization strategy. The dilution of pure OPC with amorphous silicates, and even non-cementitious fillers such as raw limestone, is now surging in popularity as the urgency and importance of reducing cement’s CO₂ footprint becomes apparent. Even so, SCMs can only partly decarbonize cement because the hydrated lime available to react with silicates and produce the desired C-S-H phase still comes from carbon-intensive OPC — and this “excess calcium” is only ~20% of the cement. Adding further SCMs beyond this point generally reduces cement strength and durability, as the cement blend ends up containing more and more inert silica “filler.”
The adoption of SCMs is a helpful decarbonization start but is insufficient without the decarbonization of lime. It also highlights another deficiency in the OPC-based route to creating C-S-H: OPC’s chemistry leaves on the table the potential strength and durability that come with a cement that uses all of the lime in the mix to optimize C-S-H formation.
However, these limitations also hint at a promising alternative approach: if it were possible to create decarbonized lime, it could be combined with SCMs or other reactive silica sources. These materials could react to create C-S-H without the need for any OPC. Such a cement would represent a modern decarbonized version of time-tested, ultra-durable ancient Roman cement.
The pressure to decarbonize cement intensifies, but (until now) there has been no way to make decarbonized lime, and hence C-S-H, without carbon capture.
The making of lime (CaO, calcium oxide, the “C” in C-S-H) is CO₂ intensive for two reasons. First, it is typically made from limestone (CaCO₃), which is nearly 50% by weight CO₂. This chemically-bound CO₂ is released when limestone is heated to high temperatures to make lime. The second source of lime’s CO₂ emissions are those from fuel combustion. Combining these two emission sources, the thermal decomposition of limestone (1) emits a total of 1.2–1.8 kg CO₂ per kg of lime produced, which translates to 0.5–0.75 kg CO₂ per kg of C-S-H produced.
The chemically-bound CO₂ in limestone would be relatively easy and inexpensive to capture if it weren’t mixed with the dilute and impure CO₂ in the furnace flue gas, the composition of which varies with the type of kiln used and the moisture of the input materials but is typically 0.4–1.0 kg CO₂/kg CaO. Portland cement production generates even more furnace CO₂ than lime production due to the greater amount of fuel needed to reach the 1,400 °C temperature at which alite is made.
The only source of “decarbonized” lime today is blast furnace slag (BFS), a lime-rich byproduct of steel making. This type of slag originates from conventional CO₂-intensive limestone that is added to blast furnaces to remove mineral impurities from the molten steel. Some carbon accounting methodologies assign blast furnace slag a CO₂ footprint equal to that of cement. The global amount of slag produced is enough for less than 5% of the world’s cement consumption, so while it is important to recycle slag into cement, slag usage will not lead to meaningful CO₂ reduction. Slag-based cement is in high demand and sometimes carries a “green premium,” despite the fact that competition for slag will not decrease global CO₂ emissions since it is available in a fixed quantity.
Decarbonizing today’s OPC kilns will require carbon capture and sequestration (CCS). However, using CCS will always increase cement’s embodied energy and cost.
The most prominent carbon capture technology for cement is amine scrubbing, a technology that uses the temperature-dependent solubility of CO₂ (an acid) in amines (bases) to capture CO₂ from dilute and impure flue gas and release it as a pure stream that can be compressed and captured. To fully decarbonize OPC production with amine scrubbing, each cement plant will require an equally sized and equally costly (~$500M per 1Mt/y cement plant) carbon capture plant on the same premises, according to a recent feasibility report and project proposal. Adding carbon capture will also approximately double the operating expenses of the cement plant, for an all-in cost between 60–200 $/ton CO₂, not including the cost of sequestering the CO₂ after capture. As a result, decarbonized cement made with amine scrubbing will have at least double the cost of today’s cement.
Further adding to the uncertainty around CCS, most amine scrubbing pilots built in the past ten years have suffered from technical and economic challenges, and alternatives to amine scrubbing are relatively immature. CCS also results in logistical complications from the lack of offtake for CO₂. The amount of CO₂ produced by the cement industry dwarfs today’s industrial uses for CO₂, so CO₂ captured from cement must be transported to a place where it can be permanently stored underground. Geologies suitable for CO₂ sequestration (depleted oil and gas reservoirs, uneconomic organic-rich deposits, saline aquifers, and salt caverns) are not universally distributed and are not always co-located with existing cement plants.
The future demands technologies that don’t emit CO₂ in the first place.
Once the goal was clear, it took us less than a year of focused effort to invent a way to make carbon-neutral lime at ambient temperature. Our design criteria were simple:
- Zero CO₂ emissions
- Cost parity with incumbent greenfield technology
- Equivalent or better technical performance
- Low-temperature operations
We developed a novel approach: ambient temperature electrochemical calcination. This breakthrough, published in Proceedings of the National Academy of Sciences in September 2019, allows us to extract calcium using low-cost electricity rather than heat. Furthermore, this approach capitalizes on the know-how, equipment, and supply chain of existing industrial electrochemical processes and has allowed us to come to market at light speed.
Our approach was based on a water-splitting electrolyzer. We split water at near-neutral pH, producing not only H₂ and O₂ but also in a pH gradient between the two electrodes (illustrated in Figure 1). Acid is co-evolved with oxygen at the anode (5), while base is co-evolved with hydrogen at the cathode (6).
(5) 2H₂O → O₂ + 4H+ + 4e-
(6) 2H₂O + 2e- → H₂ + 2OH-
A calcium-bearing mineral reacts with the acid formed at the first electrode to form dissolved calcium ions. The positively charged calcium ions migrate towards the negative electrode until they reach a pH of at least 12.5, whereupon they react with base and precipitate as solid Ca(OH)2, calcium hydroxide.
(7) Ca2+ + 2OH- → Ca(OH)₂ (s), pH >12.5
This core innovation, electrochemical calcination, began while we were still in the labs at MIT and has since been further developed at Sublime to be more energy efficient, to eliminate net production of H₂, to allow separation of CO₂ and O₂ gas streams, to extract lime continuously from the reactor, and to use commercial off-the-shelf electrolyzer hardware. However, many of the original principles remain the same.
Figure 1: Neutral-pH water electrolysis with pH-sensitive dye, showing acid (red) forming at one electrode and base (purple) at the other. In Sublime’s process, this pH gradient is used to drive the extraction and isolation of lime at ambient temperature. Photo credit: Felice Frankel.
A key driving factor for selecting this method was its capability to process any Ca-containing mineral — including ones that don’t contain CO₂— no CO₂ in, no CO₂ emissions out.
Today, Sublime’s process can make lime from a wide variety of calcium-bearing inputs. The process is naturally purifying, so impure inputs can be used, including non-carbonate calcium minerals that don’t have embedded CO₂ and low-grade limestones. We can separate the calcium from common impurities such as silica, magnesium, iron, and aluminum since these components either don’t dissolve in acid or don’t precipitate at the same pH as calcium. Unlike the process of making lime through the thermal decomposition of limestone, in which the impurities are further concentrated when half the limestone’s weight is lost as CO₂, Sublime’s process of making lime results in a pure lime almost no matter what the starting material, and other higher-value minerals, such as magnesium, can be isolated too.
Our flexible technology can use a variety of feedstocks, helping to democratize zero-emissions cement production around the world. For example, the Sublime technology can use traditional or low-grade limestone as its calcium source. Though this version of our technology produces CO₂ from the limestone as a byproduct, limestone may be needed for cement in certain locales due to a variety of geographic and economic factors. But those factors don’t spell the end of decarbonization. When limestone is used as a calcium source in our system, the CO₂ evolved is pure, cold, and already compressed to 10 bar, requiring minimal demisting and compression before it is considered captured. However, even if limestone CO₂ is vented instead of captured, and even if US grid average electricity is used instead of renewable electricity, the cement made from Sublime Lime™ has lower embodied energy than OPC and significantly lower CO₂ emissions.
When non-carbonate calcium sources are used, and if the electrolyzer is powered entirely by renewable electricity, the resulting lime is entirely carbon-neutral.
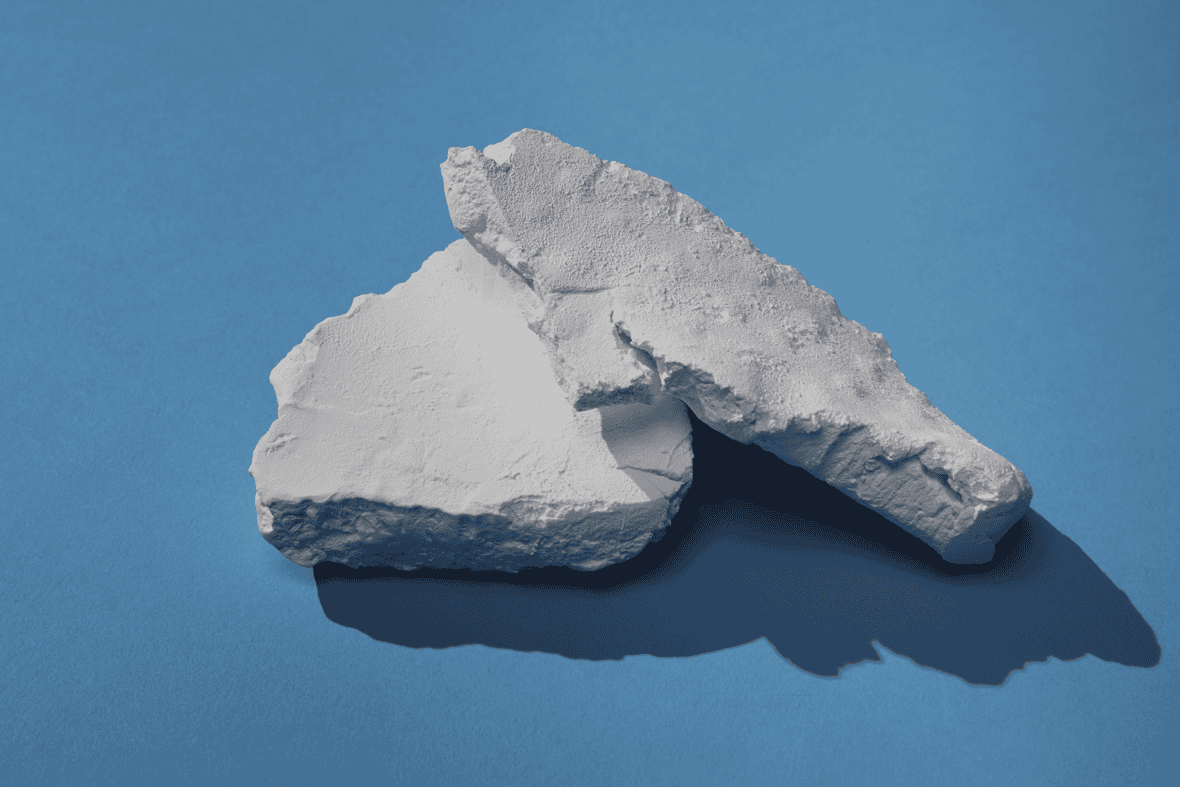
History shows that the simplest processes with the lowest embodied energy win because they are less expensive. Intuitively, we know it also makes more sense to keep fossil fuels in the ground rather than combusting them and recapturing the CO₂.
At first, Yet and I believed that the successful commercialization of our technology would result in its incorporation into the process of making OPC. Carbon-neutral Ca(OH)₂ could be used as a drop-in replacement for CaCO₃ in an OPC kiln, resulting in a 50–60% reduction in CO₂ emissions from the elimination of limestone emissions. Moreover, using hydrated lime would result in 10–15% less energy use in the kiln since Ca(OH)₂ decomposes into CaO and begins to react with silicates at a much lower temperature than CaCO₃ (550 °C rather than 850 °C). One step forward clearly came with two steps back: the addition of an electrolyzer to an OPC kiln would, like other decarbonization technologies, nearly double the CAPEX and OPEX of the cement-making process.
Because of the 1400 °C temperature required to make alite, OPC production is unlikely to ever be fully decarbonized without post-combustion carbon capture. Combustion of fuel, typically bituminous coal, is required to achieve 1400 °C. If Sublime’s technology were to be commercialized to make a truly low-carbon cement — if this technology were to be more than a Band-Aid solution, a method of making affordable low-carbon cement not only for the next decades, but also for the next millennia — Sublime’s method would have to bypass OPC and make C-S-H directly.
Today, Sublime’s goal is to make a fully decarbonized direct replacement for portland cement, which means making C-S-H, with the lowest embodied energy and the lowest CO₂ emissions.
Having created our process for manufacturing decarbonized lime, we had the key to creating a decarbonized cement. Sublime Cement™ relies on the reaction of our decarbonized lime with reactive silica, which can be obtained from a wide variety of low-cost, abundant natural minerals or industrial waste materials. Sublime’s team has carefully designed and tested hundreds of prototype cements to validate this approach. Now Sublime Cement™ hardens even stronger than pure OPC. It also matches OPC’s workability, setting time, and other properties that impact concrete producers, making it a drop-in replacement for today’s portland cement. Since it can be made with decarbonized electricity and non-carbonate feedstock materials, Sublime Cement™ has significantly lower embodied CO₂ than OPC. Currently, Sublime Cement is 70% decarbonized and on the path to near-zero-carbon by 2030.
The construction industry is responding to the call for a decarbonized future.
Cement companies, concrete companies, structural engineers, architects, developers, and perhaps most importantly, infrastructure owners such as corporations and governments are mobilizing to decarbonize the built environment. Nearly every cement company has committed to being net zero by 2050. Ready-mix concrete companies such as Central Concrete/Vulcan, Ozinga, and Stoneway, among others in the US, are pioneering low-carbon concrete blends. The American Concrete Institute (ACI) has established a center of excellence, NEU, to facilitate the validation and adoption of new low-carbon cement. ASTM International has developed performance-based standards that give structural engineers the freedom to specify non-OPC-based cements, so long as they pass rigorous performance tests. The 2006 initiative of Architecture 2030 has been adopted by most of the world’s largest and most influential architecture firms, representing over $100 billion in construction annually. Similarly, over a hundred structural engineering firms in the US have banded together to form Structural Engineers 2050 (SE2050), which aims to achieve net zero embodied carbon in structural systems by 2050 by using sustainable structural materials.
Demand for low-carbon cement is growing.
Building owners’ preferences are among the most important drivers of change in the construction industry. A recent study found that 94% of engineers and contractors reported that their clients are requesting reductions in embodied carbon in their projects. At the forefront of this trend is the First Movers Coalition, a public-private partnership to commercialize clean technologies through advanced purchase agreements. The group includes 65 prominent companies and ten government partners committed to buying at least 10% near-zero-carbon cement by 2030. In total, FMC members have pledged $12B, the world’s largest demand signal for ultra-low-carbon products.
Sublime is on track to produce 1 million tons per year of low-carbon cement by 2028.
We’re committed to scaling up the technology quickly and safely. We’re currently operating our pilot plant, which can produce up to 100 tonnes per year of cement. Next year, we will start construction of our commercial demonstration plant, which will produce tens of thousands of tonnes of cement per year. After extensive months-long diligence, we have already shortlisted a handful of locations for this plant. We will operate this plant in 2025 and prepare a data package for partners and investors to enable a full-scale, million-tonne-per-year cement plant built in 2026/2027 and operating by 2028.
We have a singular focus: to swiftly, substantially, and permanently reduce anthropogenic CO₂ emissions through decarbonizing cement production.
In the past 200 years since the industrial revolution, humanity’s nascent knowledge of chemistry has been wielded with devastating effects on ecosystems and communities. But now, with 200 years of innovation and discovery behind us, we have the power to right those wrongs without losing what we’ve gained. Advances in science and technology have increased our understanding and appreciation for the intricacies of our planet, while also providing us with the tools to innovate and implement sustainable industrial practices.
We are committed to partnering with individuals and organizations that share our vision of deep decarbonization in the cement industry by 2050.
Achieving this ambitious goal requires collaboration and innovation, and we are committed to partnering with concrete producers, cement companies, contractors, engineers, architects, infrastructure owners, and policymakers who share our determination to make a positive impact on the world. We approach our work with courage, integrity, and a willingness to learn, and we are always looking for team members who take pride and find joy in this important work.